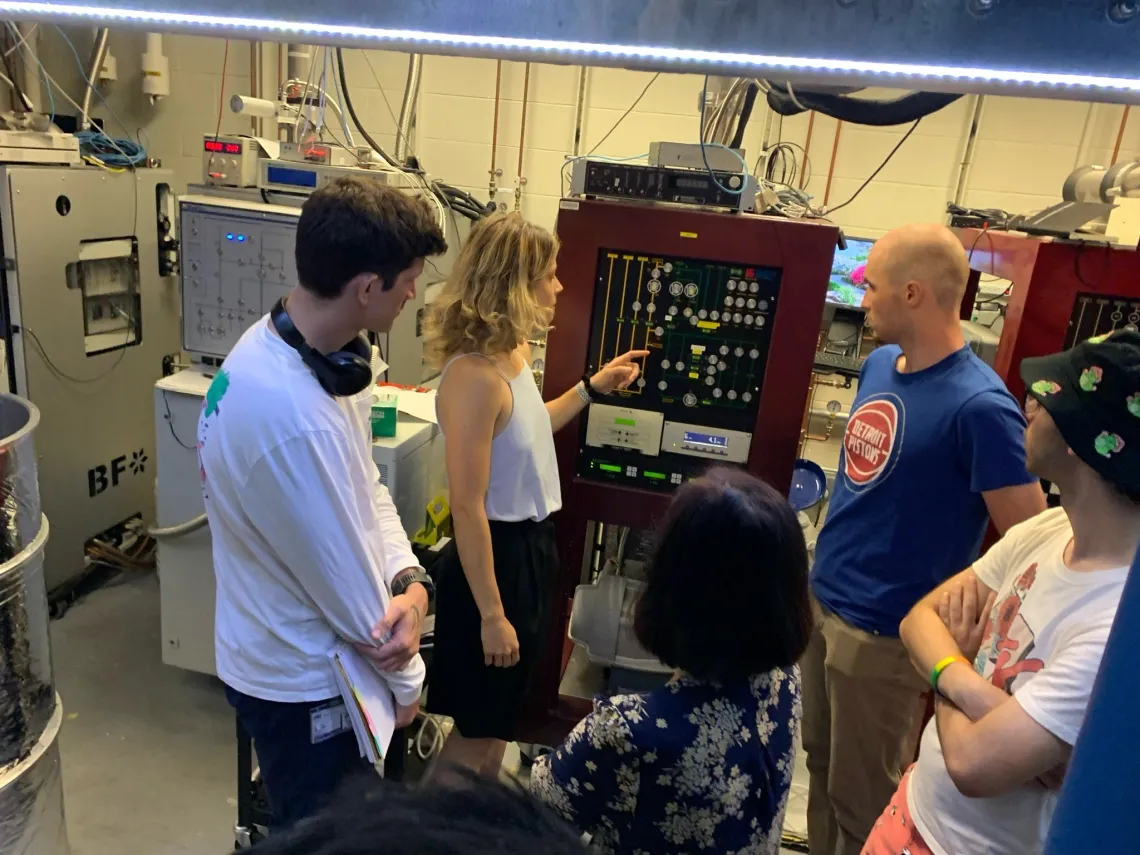
.
Click each row to expand:
The University of Arizona is the lead institution for the NSF-ERC Center for Quantum Networks (CQN) which supports a research thrust focused on Societal impact of quantum technologies. As part of our societal impacts research for quantum technologies, we are working to ensure that the society is well prepared for broad, affordable, and equitable access to the quantum internet, the associated applications, and their economic implications. We are studying the social and policy implications of this budding technology. To do this, we support social science research on the legal, economic, and social implications of quantum communications. Example research areas being explored (supported as part of CQN's quantum society fellowship program, and other projects) are:
- Quantum Internet standards and governance,
- Quantum technology marketplace,
- Post-quantum privacy and security, and
- Equitable access, fairness, and accountability.
Also see: Quantum applications roadmap
We have a strong interdisciplinary research team focused broadly on problems surrounding quantum communications and networking. We are studying: (1) the use of quantum resources in improving classical communications, (2) designing systems that would allow quantum communications (transmitting qubits) over long distances, and (3) theoretical foundations and protocols for secure and covert communications with security guarantees sought for a future-proof quantum-equipped adversary.
- Classical communications: AQuI researchers are looking into quantum-enhanced receiver designs for higher communications capacity (quantum enhancements are most prominent in the low received photon flux regime), and using pre-shared entanglement to enhance classical communications rates (quantum enhancement are most prominent in the regime of high noise, i.e., long wavelength communications, and low transmitter brightness). This research thread includes the study of information theoretic limits, designs of quantum-enhanced transmitters and receivers, quantum-circuit-realizations of quantum-optimal receivers, and error correction codes. In experimental work, we are designing and building joint-detection receivers that could attain "superadditive" communications capacity---higher reliable-communications data rates attainable than any classical/conventional optical receiver.
- Quantum communications: We are studying information theoretic limits (e.g., quantum channel and network capacity), quantum error correction codes (for example, for entanglement distillation and quantum network coding for multiparty communications), system designs involving spin-photon interfaces (for efficient transduction of matter qubits to photonic qubits and vice versa), high-rate high-fidelity photonic entanglement sources, and end-to-end quantum repeater and quantum network architectures for long-distance reliable communications of quantum information. We are developing algorithms and policies for switching and routing of entanglement in a distributed quantum network, evaluating rate regions for simultaneous distribution of different forms of entanglement, and more. We are developing a quantum network testbed in our campus, connecting multiple buildings and laboratories within them, which is equipped with color-center spin-based quantum memories, efficient spin-photon interfaces, superconducting nanowire single photon detectors, and photonic entanglement sources, optical fiber, switches, and other standard telecommunications equipment.
- Secure and covert communications: One of the most cited applications of quantum communications as a service, is to enable provably secure communications; classical data communications that cannot be decoded by an adversary who taps the line, but in a way such that the security guarantees stem from the laws of physics as opposed to our perceived hardness of certain math problems such as factoring or the discrete log (which classical cryptographically-secure algorithms' security promises rely upon). AQuI researchers are studying protocols, methods and demonstrations of various offshoots, applications and variants of quantum-secured communications. Examples include: covert communications and sensing --- designing communications and sensing systems where the presence of the transmission itself is hidden from a quantum equipped adversary, secure multiparty computation algorithms, piggybacking secure information on covert information and more. On our campus testbed, we are working on demonstrating several quantum security protocols connecting multiple building and laboratory sites.
There are multiple research threads we are pursuing on various quantum materials and devices, which will contribute towards building functional quantum systems. These include:
- Color center quantum memories: we are fabricating, testing and building up systems comprising of both bulk color centers and color centers integrated with photonic waveguides, for building quantum repeaters and also for other fundamental research projects such as using color centers as a mediator for microwave-to-photon transduction, color center arrays to be used to generate photonic cluster states, and more. We are also working on efficient and scalable spin readouts, and microwave spin control methods, e.g., using tailored strain induced methods.
- Single-photon detector arrays: We are researching integrating superconducting nanowire single photon detectors on integrated photonic chips, for various on-chip processing tasks, ranging from heralded entanglement swaps and other complex quantum measurements to entangle waveguide-integrated spin memories, to generating non-Gaussian states of light such as GKP qubits by photon detection on multimode squeezed states of light on chip.
- All-photonic cluster states: We are working on quantum repeater architectures where the role of (matter-based) quantum memories are replaced by photonic cluster states, both of "discrete variable" and "continuous variable" photonic qubit varieties, which protect against photonic loss seen as a quantum error mechanism. We are working on scalable on-chip generation of multimode squeezed states, and programmable spectral-spatial linear transformations to manipulate such states, in order to generate the said cluster states.
- Cold atoms: We are working on laser cooled and trapped neutral atoms as qubits and using our testbed to study new techniques for quantum state preparation, coherent control, quantum tunneling and quantum transport phenomena in optical lattices. Research includes studying macroscopic superposition states and coherent evolution in dissipative quantum systems, techniques for laser cooling, trapping and manipulation of atoms and ions, and using our testbed to uncover mysteries of quantum dynamics in a small yet robust Hilbert space of a few qubits.
- Superconducting qubits: We are building up capability in superconducting qubits, with a research focus on quantum transduction (spanning microwave, spin, phonon and photonic degrees of freedom), photonic-heralded generation of remote entanglement among superconducting qubits.
- Opto-mechanical quantum systems: We are working on various aspects of quantum opto-mechanical systems, studying for instance: radiation pressure effects, noise in optical interferometers, quantum measurement and control, nanomechanical devices as macroscopic quantum systems, interactions between ultra-high-Q optical and mechanical resonators, and cryogenic optomechanics.
Quantum enhanced sensing, imaging and metrology is a large part of our research portfolio. Various AQuI faculty members have expertise in sensor technologies, ranging:
- Bio-imaging techniques such as fluorescence microscopy, multi-photon imaging, and endoscopic imagers,
- Magnetic field sensing using spin-based sensors in an atomic defect array,
- Quantum-enhanced astronomical imaging using pre-detection spatial and/or temporal/spectral mode transformations for super-resolution imaging and object detection / classification problems,
- Opto-mechanical sensors, e.g., nano-trampoline networks enhanced by entangled squeeze light, for sensing gravitational waves and dark-matter search,
- Quantum-enhanced radars for stand-off target detection and classification for application, e.g., for microwave operation in the stealth mode, and more.
AQuI faculty is engaged in quantum sensing research spanning: fundamental theory (e.g., new tools in multiparameter quantum Bayesian estimation theory), applied theory (e.g., finding designs of optimal probes and receiver designs for certain imaging tasks), device design and fabrication (e.g., development of squeezed light sources, building mode sorters, designing and building phonon-photon interfaces, and more), and experimental demonstrations of quantum enhanced sensors.
The following aspects of quantum computing are being researched by AQuI faculty, students and postdocs:
- Fault-tolerant quantum error correction. The University of Arizona, through its partnership with the DoE SQMS center Superconducting quantum materials and systems, and through multiple UArizona-led research projects, we are studying many aspects of fault-tolerant error correction for quantum computing:
- Quantum LDPC codes, iterative decoding such as message passing based algorithms, and performing fault-tolerant gates,
- Encoders and decoders for codes that function despite the quantum logic used within them are noisy,
- Error correction for protection of loss/erasure errors versus Pauli-like errors, for applications to measurement based quantum computing and cluster state based quantum repeaters,
- Quantum convolutional codes and decoders, and more.
- NISQ. AQuI faculty are also engaged in some research in Noisy Intermediate Scale Quantum (NISQ) quantum algorithms, including: (1) using quantum-enhanced samplers for problems in graph theory, (2) small quantum computers used as quantum-optimal photonic receivers, e.g., for communications and sensing problems, and (3) quantum machine learning algorithms applied to problems in photonic sensing.
- Superconducting qubits. We are setting up a state of the art Superconducting Qubit laboratory in the GCRB, where our research focus will include remote entanglement generation among superconducting qubits, and using superconducting qubit based logic for demonstrations of quantum operations such as entanglement distillation and error correction. We will also pursue superconducting to photon transduction, e.g., via phonon-mediated interactions, in our superconducting qubit laboratory.